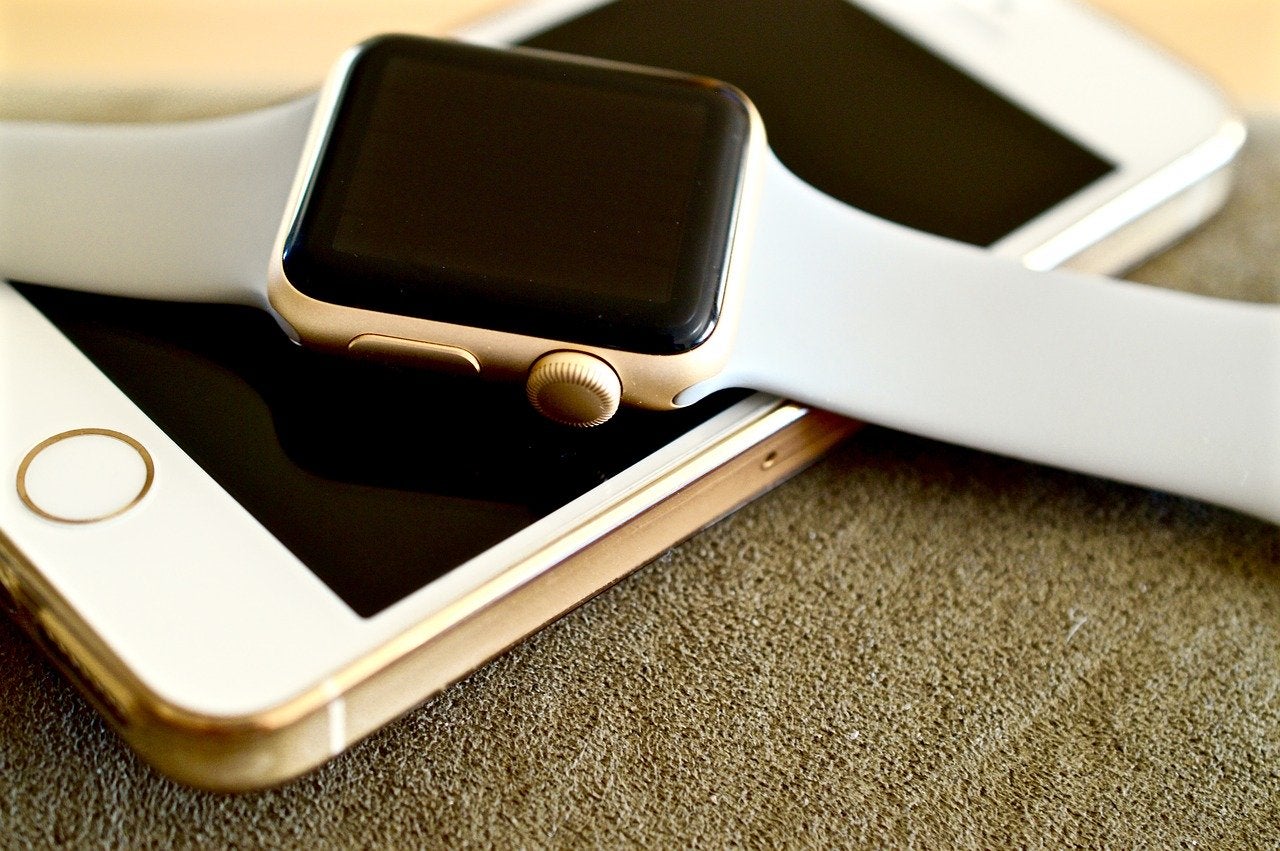
Able to be compressed, twisted and conformed to uneven surfaces without losing functionality, stretchable electronics have captured the imagination of engineers in a host of different industries. In healthcare, the elasticity of polymers such as silicone has given rise to emerging technologies that can be made to mimic the behaviour of skin. Jim Banks explores their potential with Matt Pharr, assistant professor of mechanical engineering at Texas A&M University.
Whether we call them elastic circuits, elastronics or stretchable electronics, there is an ever-growing family of devices that are challenging traditional views of how computer circuits can operate. Usually, the sensitive electronic components of computers, sensors and other technologies are held within hard outer casings to protect them from damage. Now, they are being incorporated into devices that can stretch with the skin.
Stretchable electronics combine silicon-based computer circuits with flexible substrates – frequently made from silicone or polyurethane – which can be distorted without impacting the functionality of the circuitry. In their simplest form, the familiar components of a printed circuit board are placed on or embedded in an elastomer, but the interaction of rigid interconnections and flexible substrates generates many challenges.
The potential benefits such devices offer, however, has led to rapid development of the technology. One key application is in energy storage, with stretchable electronics using double-layer supercapacitors to enable dynamic charging and discharging.
“When stretched, some polymers can generate electrical energy,” explains Matt Pharr, assistant professor of Mechanical Engineering at Texas A&M University. “Also, a dielectric elastomer can be used as an activator, as it flexes when a voltage is applied. But in the medical sphere, a more common application is the measuring of biomarkers such as those from hydration through the collection and analysis of sweat.”
Energy harvesters use dielectric elastomers, which are smart materials that belong to the group of electroactive polymers. Dielectric actuators are lightweight and have a high elastic energy density, and they can transform electric energy into mechanical work. It is in medicine, however, that the potential for innovation is greatest.
“One very hot area of research is in the biomedical industry,” says Pharr. “Some professors have already spun out companies with technology such as a flexible patch that can be bent, though not stretched, and can conform to the shape of the body. The patch can measure ECG, muscle activation, temperature or even kinematic signals such as where you are and how fast you are travelling.”
Stretchable electronics in the healthcare sector
A strong focus for Pharr’s research at Texas A&M’s Experimental Solid Mechanics Laboratory has been the development of materials for energy storage and conversion, the deformation and fracture of soft materials, and the mechanics of flexible or wearable electronic devices. As a result of his work, Pharr is seeing the healthcare sector engage in considerable research into areas such as diagnostics, surgery and the treatment of specific conditions such as diabetes.
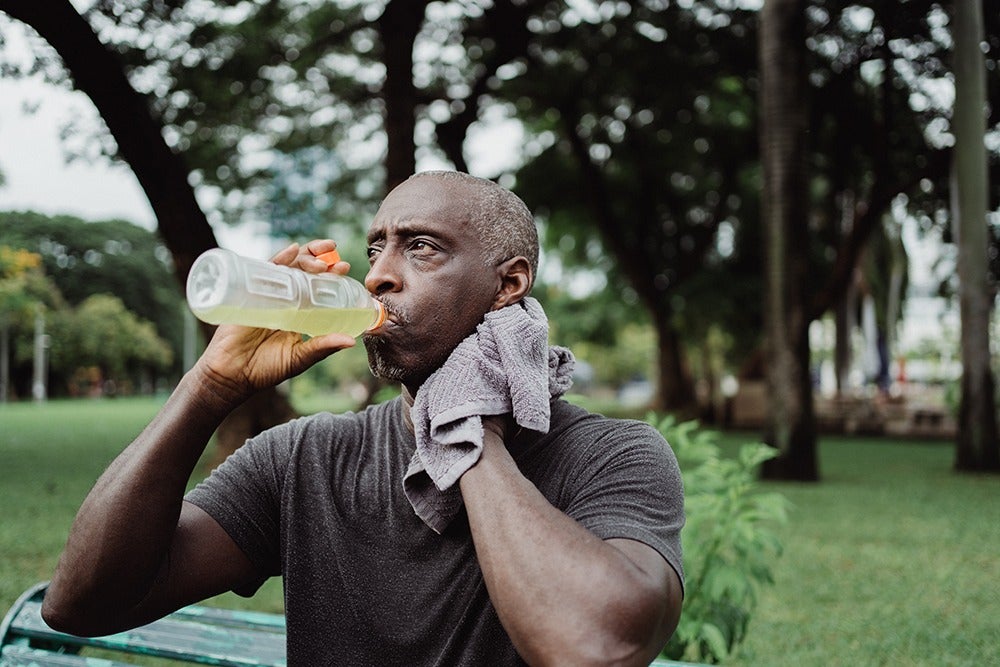
In diagnostics, wearable devices can interact seamlessly with the body, enabling the non-invasive detection of diseases. For diabetes, wearable patches can increasingly detect accurate blood glucose levels in sweat and, potentially, deliver the required medicine on demand. In surgery, stretchable electronics are the foundation for the development of soft robots, which could perform procedures that are minimally invasive and highly precise.
Wearable monitors have been getting a lot of airtime. One example is the advertising campaign by Gatorade, which features a sweat-reading wearable device. The Gatorade skin patch, which has been developed in partnership with Northwestern University, alerts wearers if they are dehydrated by monitoring electrolyte levels in their sweat.
The transparent silicone patch, which is roughly the size of a coin, can be placed almost anywhere on the body and is flexible like a sticking plaster. The device channels sweat through microfluidic channels, where it passes through dye, the colour of which gives an indication of the levels of chloride contained in the sweat.
“Biomarkers from sweat that are used to measure fatigue or hydration are very small in size, so we need to calibrate for small quantities and background noise,” remarks Pharr. “We could use carbon nanotubes and elements that combine with the biomarkers to change their conductivity, so a lot of work is being done in those areas now.”
Dehydration is not, however, the only biometric that can be tracked, though for more sophisticated sweat-monitoring systems to work effectively they must be highly sensitive. Nor is sweat the only medium through which measurements can be taken.
“From the therapy perspective, I worked in collaboration with the University of California, San Diego (UCSD) on a technology designed for monitoring organs inside the body,” Pharr says. “It is a wearable version of a sonogram for the acoustic imaging of internal organs. Ultimately, this technology could be used to stimulate those organs for therapy purposes, in the same way that a heating pad might be worn anywhere on the body.”
“A lot of papers published every year on wearables and thousands of companies are focusing on it,” adds Sheng Xu, assistant professor of nanoengineering at UCSD, who worked with Pharr on the project. “The Apple Watch and similar devices have an LED light that can penetrate a few millimetres, but my group is the first to look at signals deep beneath the skin using a wearable device. It is a wearable ultrasound patch, and ultrasound can see through the body as if looking through glass.”
Commercial challenges
Inevitably, the more sensitive and sophisticated a device, the more challenging it is to make it work. Adhesion is one challenge and power sources present another hurdle, as commercial batteries are not very biocompatible. Furthermore, the device must not limit the wearer’s ability to move, so the goal is to create a small device that stretches with the skin and does not irritate it.
Devices incorporating stretchable electronics could be highly effective in two very high-impact applications. Almost five million people in the UK and countless millions more worldwide suffer from diabetes, so a small, flexible device worn on the skin that measures blood glucose would be a great boon to their treatment regimes. Hypertension kills more people than all cancers combined, so a wearable device to continuously monitor blood pressure would be highly advantageous.
“Traditional electronics are based on rigid elements,” notes Pharr. “Therefore, the stretchable part is not the conductive part. Instead, traditional electronics are being integrated with the stretchable elements. For example, metals are being placed on a silicone-based polymer. If the device is well designed, then the only part that stretches is the polymer.”
“What we are working on now is making the stretchable polymers conductive, but their conductivity is orders of magnitude worse than the metal components,” Pharr adds. “There is a lot of research happening now on conductive polymers, but the technology is not there yet.”
Xu is working on wearable devices that are capable of acquiring multichannel physiological signals from the human body, and his search of pliable building blocks requires bridging the gap between conventional high-performance hard materials and soft biology. His goal is to design a matrix that hybridises hard materials with soft substrates, so that the local rigid elements are located in islands along the substrate, making the device soft and flexible overall.
Stress and repeated stretching
Pharr’s group at the Experimental Solid Mechanics Laboratory is working on strategies for making devices that are mechanically robust if they are stretched many times. Examination under stress loading is informing the design of substrates that can reduce the stress on the electronic components when in use.
“The issue is the elasticity or stiffness of the components,” Pharr remarks. “Silicone elastomers can perform in a way that is similar to the skin, so we can integrate electronics, but the electrical components are rigid, so if we integrate them in a high density then it can make the device heavy or uncomfortable to wear, or they may make it less flexible and more likely to fall off.
“One solution could be to have a layer of silicone elastomer on the skin, then an ionic liquid layer,” Pharr adds. “Liquid flows, so it does not pull on the elastomer or the metal layer above it, which is decoupled from the forces of motion from the movement of the body or the skin.
A big breakthrough would come if you could make the stretchable components have greater conductive functionality. There are conductive polymers but their performance is not great,” he adds.
Undoubtedly, the number of potential applications of stretchable electronics will continue to expand rapidly. For Pharr, Xu and many others working in this field, the promise of devices that can mimic the skin or, more enticingly, be flexible enough to work inside the body, is enough to ensure that the future of many medical devices will increasingly be flexible in form.
This article originally appeared in Volume 1, 2020, of Medical Device Developments. The full issue can be viewed here.